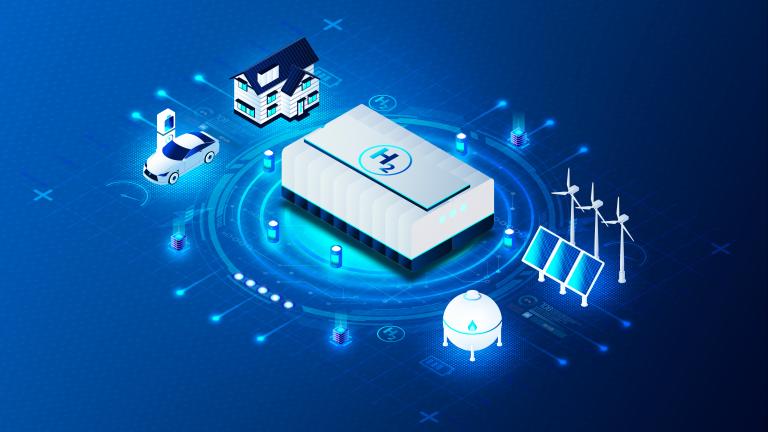
In the intensifying debate about alternative pathways for rapid decarbonization, hydrogen is increasingly viewed as a critical building block for storing and flexibly dispatching large amounts of carbon-free energy1;2. Among alternative hydrogen production technologies, Power-to-Gas (PtG) in the form of electrolytic hydrogen has received particular attention3–5. Large-scale deployment of these technologies, however, is generally expected to hinge on substantial cost declines and energy conversion improvements. To accelerate the pace of these improvements, governments around the world have recently introduced sizeable regulatory initiatives and subsidy programs for the development, deployment, and manufacturing of hydrogen equipment6;7.
This paper projects cost and conversion efficiency improvements for three prevalent PtG technologies: alkaline, polymer electrolyte membrane (PEM), and solid oxide cell (SOC) electrolysis. Our analysis is grounded in a learning-by-doing model that postulates that system prices for electrolyzers and their conversion efficiency decline at a constant rate with every doubling of cumulative installments of the technology in question. Such learning models have proven highly descriptive in the context of solar photovoltaics, onshore wind turbines, or lithium-ion batteries. Scarcity of data has so far limited the estimation of learning curves to alkaline electrolysis or to a single equipment manufacturer. Some earlier studies estimate the rate of past cost declines of PtG technologies against time or rely on expert opinions about future cost developments.
Our analysis provides a comprehensive assessment of the dynamics in system prices and energy efficiency for the three PtG technologies by tracking global observations on investment expenditures and energy consumption. This information is linked to capacity installations at facilities commissioned worldwide between 2000–2020. Our estimates return significant and robust learning curves for system prices in the range of 83–86% (Figure 1). Thus, system prices declined by 14–17% compared to the price levels prior to the doubling of cumulative installments. The relatively young SOC technology is projected to show the sharpest price decline at a 17% learning rate. PEM electrolyzers, in contrast, have experienced high capacity growth and a rapid price decline between 2003 and 2020. Here, our estimates yield a relatively slow learning rate of 14%. For conversion efficiency, we estimate that every doubling of cumulative installed capacity reduces the required kilowatt-hours (kWh) per kilogram (kg) of hydrogen produced by approximately 2% across all three technologies.
Figure 1: Estimates of learning curves. This figure plots the global system prices in 2020 $US against the global cumulative installed capacity together with our estimates of the corresponding learning curves for (a) alkaline, (b) PEM, and (c) SOC electrolyzers. The figure also plots the energy consumption against the global cumulative installed capacity together with our estimates of the corresponding learning curves for (d) alkaline, (e) PEM, and (f) SOC electrolyzers. Areas shaded in red represent 95%-confidence intervals.
Our regression results can be extrapolated to yield forecasts for the system prices and conversion efficiencies of the three PtG technologies in question by the year 2030. Even for divergent growth forecasts issued by different industry and policy sources, the extrapolated values fall into a relatively narrow range. Specifically, our calculations project ranges for system prices by 2030 of $285–475/kW for alkaline, $225–352/kW for PEM, and $441–767/kW for SOC electrolysis. Regarding the energy consumption of PtG systems, our projections for 2030 yield ranges of 47–49 kWh/kg for alkaline, 47-50 kWh/kg for PEM, and 36–38 kWh/kg for SOC technology. Compared to earlier estimates articulated by industry experts, technical reports, and academic studies, our projections are consistently and substantially below most earlier estimates. While this can be attributed to multiple factors, the most important one is that our projections model technological progress not as an exogenous function of time but as an endogenous process driven by deployment rates.
Recognizing the potential of hydrogen as a decarbonized energy source, the U.S. Department of Energy7 articulated the Hydrogen Shot initiative in 2021. According to this initiative, the cost of producing hydrogen is to come down to $1.0/kg by the year 2030. The system prices and conversion efficiencies we forecast are useful in gauging whether the U.S. Department of Energy’s goal appears to be a “long shot.” Depending on the growth of capacity installations, our calculations yield estimates for the life-cycle cost of electrolytic hydrogen production in the range of $1.6-1.9/kg by 2030. These findings lead us to conclude that the Hydrogen Shot target by the U.S. Department of Energy of producing clean hydrogen at a cost of $1.0/kg by 2030 is ambitious but not unrealistic. Because electricity prices will become the dominant component of the life-cycle cost of hydrogen by 2030, the attainment of the Hydrogen Shot target via electrolytic hydrogen ultimately hinges on the availability of inexpensive and clean electricity.
Our findings on the economics of electrolytic hydrogen speak directly to several recent policy initiatives. We first note that even our most ambitious growth scenario for electrolyzer deployment falls significantly short of the target for 2030 by the International Energy Agency (IEA). As part of its “Net-zero by 2050” scenario, the IEA8 postulates 850 GW of installed capacity by 2030 and 3,000 GW by 2045. Furthermore, most data points underlying our projections were set prior to the recent hydrogen initiatives by the European Union and the Inflation Reduction Act in the United States. The production tax credit of up to $3.0/kg of clean hydrogen available under the Inflation Reduction Act is likely to advance the deployment growth of PtG systems significantly in the United States. This growth will be reinforced by the goal of the European Union, which seeks to induce its member states to collectively produce 20 million tons of green hydrogen annually by the year 2030.
References
[1] Staffell, I. et al. The role of hydrogen and fuel cells in the global energy system. Energy & Environmental Science 12, 463–491 (2019).
[2] Davis, S. J. et al. Net-zero emissions energy systems. Science 9793 (2018).
[3] Sepulveda, N. A., Jenkins, J. D., Edington, A., Mallapragada, D. S. & Lester, R. K. The design space for long-duration energy storage in decarbonized power systems. Nature Energy (2021).
[4] Guerra, O. J. et al. The value of seasonal energy storage technologies for the integration of wind and solar power. Energy & Environmental Science (2020).
[5] Ueckerdt, F. et al. Potential and risks of hydrogen-based e-fuels in climate change mitigation. Nature Climate Change (2021).
[6] Repowereu: A plan to rapidly reduce dependence on Russian fossil fuels and fast forward the green transition (2022).
[7] U.S. Department of Energy. Secretary Granholm launches hydrogen energy earthshot to accelerate breakthroughs toward a net-zero economy (2021).
[8] International Energy Agency. Net zero by 2050 - a roadmap for the global energy sector.
Further Reading:
CEEPR WP 2023-09
About The Authors
![]() |
Gunther Glenk is an Assistant Professor for Business at the University of Mannheim. His research examines questions related to corporate transitions toward zero net emissions. Specific topics include the economics and management of corporate carbon emissions, decarbonization and sustainable energy technologies, and incentives for climate action. Recent work has focused on the competitiveness of clean energy technologies, such as energy storage, renewable hydrogen, and electric mobility. Professor Glenk received his B.Sc., M.Sc., and Doctorate in Management and Technology from the Technical University of Munich. |
![]() |
Philip Holler is a doctoral candidate at the Mannheim Institute for Sustainable Energy Studies (MISES) and part of the Graduate School of Economic and Social Sciences at the University of Mannheim. His research focuses on the competitiveness of low-carbon technologies, in particular energy storage and green hydrogen. Holler received his B.Sc. and M.Sc. in Management from the University of Mannheim. |
![]() |
Stefan Reichelstein is the William R. Timken Professor of Accounting, Emeritus at Stanford Business School, and director of the Mannheim Institute for Sustainable Energy Studies (MISES). His research focuses on cost and profitability analysis, decentralization, internal pricing, and performance measurement. His research projects span analytical models, empirical work, and field studies. Insights from his research have been applied by a range of corporations and government agencies. In recent years, he has also studied the cost competitiveness of low-carbon energy solutions with a particular focus on solar PV and carbon capture by fossil fuel power plants. Professor Reichelstein received his Ph.D. from the Kellogg School of Management at Northwestern University in 1984. Prior to that, he completed his undergraduate studies in economics at the University of Bonn in Germany. |